The reflex circuit represents the basic structure by which the mammalian central nervous system senses and responds to peripheral stimuli. Accurate modeling of this system in a controlled in vitro environment would therefore be beneficial for studying neural circuits, their development, the establishment of wiring specificity in reflex circuits, and the specific mechanisms controlling the functionality and regulation of these structures. Such a model system may also be beneficial for improving our understanding of spinal cord injuries, diseases, and treatments, as well as providing a useful test-bed for the development of novel therapeutics.
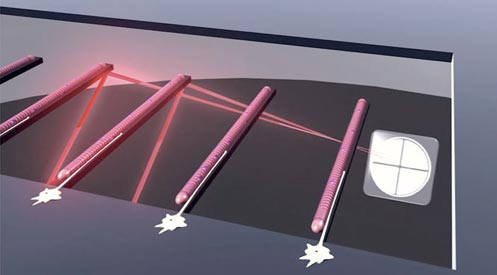
Our group is currently funded to develop an integrated in vitro model of the reflex arc capable of measuring the functional outputs of the cultured cells in real-time. As part of this work, we have demonstrated the ability to isolate and maintain sensory neurons[7], motoneurons[8] and both intra-[9] and extrafusal[8, 10, 11] skeletal muscle fibers from primary rodent sources in defined, serum-free conditions. Use of microscale silicon cantilevers and modified AFM technology facilitates the assessment of the contractile function of cultured skeletal muscle in a non-invasive manner[12, 13]. Physical deformation of cantilevers supporting intrafusal fibers likewise enables activation of these stretch-responsive cells in order to evaluate the sensory portion of the reflex arc[9]. Furthermore, data collected by our group has demonstrated the ability of these cells to form physiologically accurate synaptic structures in vitro[7, 9, 14, 15], highlighting the suitability of these cultures for developing neuronal circuits.
Current efforts are focused on the integration of these cell types into a single chip incorporating all aspects of the reflex arc. We are also attempting to transition this model into one capable of supporting human cells, from both healthy and diseased patients, in order to greatly improve the clinical relevance of this work.
References
[1] Esch MB, Smith AS, Prot JM, Oleaga C, Hickman JJ, Shuler ML. How multi-organ microdevices can help foster drug development. Advanced drug delivery reviews. 2014.
[2] Smith AST, Long CJ, Pirozzi K, Hickman JJ. A functional system for high-content screening of neuromuscular junctions in vitro. Technology. 2013;1:37-48.
[3] Sung JH, Esch MB, Prot JM, Long CJ, Smith A, Hickman JJ, et al. Microfabricated mammalian organ systems and their integration into models of whole animals and humans. Lab on a chip. 2013;13:1201-12.
[4] Smith AS, Long CJ, Berry BJ, McAleer C, Stancescu M, Molnar P, et al. Microphysiological systems and low-cost microfluidic platform with analytics. Stem cell research & therapy. 2013;4 Suppl 1:S9.
[5] Guo X, Ayala JE, Gonzalez M, Stancescu M, Lambert S, Hickman JJ. Tissue engineering the monosynaptic circuit of the stretch reflex arc with co-culture of embryonic motoneurons and proprioceptive sensory neurons. Biomaterials. 2012;33:5723-31.
[6] Rumsey JW, Das M, Bhalkikar A, Stancescu M, Hickman JJ. Tissue engineering the mechanosensory circuit of the stretch reflex arc: Sensory neuron innervation of intrafusal muscle fibers. Biomaterials. 2010;31:8218-27.
[7] Wilson K, Das M, Wahl KJ, Colton RJ, Hickman JJ. Measurement of contractile stress generated by cultured rat muscle on silicon cantilevers for toxin detection and muscle performance enhancement. PLoS ONE. 2010;5.
[8] Das M, Rumsey JW, Bhargava N, Stancescu M, Hickman JJ. A defined long-term in vitro tissue engineered model of neuromuscular junctions. Biomaterials. 2010;31:4880-8.
[9] Das M, Rumsey JW, Bhargava N, Gregory C, Riedel L, Kang JF, et al. Developing a novel serum-free cell culture model of skeletal muscle differentiation by systematically studying the role of different growth factors in myotube formation. In Vitro Cell Dev Biol Anim. 2009;45:378-87.
[10] Wilson K, Molnar P, Hickman JJ. Integration of functional myotubes with a Bio-MEMS device for non-invasive interrogation. Lab on a chip. 2007;7:920-2.
[11] Das M, Gregory CA, Molnar P, Riedel LM, Wilson K, Hickman JJ. A defined system to allow skeletal muscle differentiation and subsequent integration with silicon microstructures. Biomaterials. 2006;27:4374-80.
[12] Das M, Molnar P, Devaraj H, Poeta M, Hickman JJ. Electrophysiological and morphological characterization of rat embryonic motoneurons in a defined system. Biotechnology progress. 2003;19:1756-61.
[13] Stenger DA, Hickman JJ, Bateman KE, Ravenscroft MS, Ma W, Pancrazio JJ, et al. Microlithographic determination of axonal/dendritic polarity in cultured hippocampal neurons. Journal of neuroscience methods. 1998;82:167-73.
[14] Ravenscroft MS, Bateman KE, Shaffer KM, Schessler HM, Jung DR, Schneider TW, et al. Developmental Neurobiology Implications from Fabrication and Analysis of Hippocampal Neuronal Networks on Patterned Silane-Modified Surfaces. Journal of the American Chemical Society. 1998;120:12169-77.
[15] Schaffner AE, Barker JL, Stenger DA, Hickman JJ. Investigation of the factors necessary for growth of hippocampal neurons in a defined system. Journal of neuroscience methods. 1995;62:111-9.
[16] Hickman JJ, Bhatia SK, Quong JN, Shoen P, Stenger DA, Pike CJ, et al. Rational pattern design for in vitro cellular networks using surface photochemistry. Journal of Vacuum Science & Technology A: Vacuum, Surfaces, and Films. 1994;12:607-16.
[17] Stenger DA, Pike CJ, Hickman JJ, Cotman CW. Surface determinants of neuronal survival and growth on self-assembled monolayers in culture. Brain Research. 1993;630:136-47.
[18] Stenger DA, Georger JH, Dulcey CS, Hickman JJ, Rudolph AS, Nielsen TB, et al. Coplanar molecular assemblies of amino- and perfluorinated alkylsilanes: characterization and geometric definition of mammalian cell adhesion and growth. Journal of the American Chemical Society. 1992;114:8435-42.